Theoretical principles for biology: organization
Progress in Biophysics and Molecular Biology
In the search of a theory of biological organisms, we propose to adopt organization as a theoretical principle and define it as closure of constraints.
Abstract
Abstract In the search of a theory of biological organisms, we propose to adopt organization as a theoretical principle. Organization constitutes an overarching hypothesis that frames the intelligibility of biological objects, by characterizing their relevant aspects. After a succinct historical survey on the understanding of organization in the organicist tradition, we offer a specific characterization in terms of closure of constraints. We then discuss some implications of the adoption of organization as a principle and, in particular, we focus on how it fosters an original approach to biological stability, as well as and its interplay with variation.
Keywords: Theoretical principle, Organization, Constraints, Closure, Stability, Organicism
Table of contents
Reading time: ~78 min
Theoretical principles for biology: organization
aInstitut d’Histoire et de Philosophie des Sciences et des Techniques (IHPST) - UMR 8590, 13, rue du Four, 75006 Paris, France
b Laboratoire "Matière et Systèmes Complexes" (MSC), UMR 7057 CNRS, Université Paris 7 Diderot, 75205 Paris Cedex 13, France
c Centre Cavaillès, République des Savoirs, CNRS USR3608, Collège de France et École Normale Supérieure, Paris, France
dDepartment of Integrative Physiology and Pathobiology, Tufts University School of Medicine, Boston, MA USA
Abstract
In the search of a theory of biological organisms, we propose to adopt organization as a theoretical principle. Organization constitutes an overarching hypothesis that frames the intelligibility of biological objects, by characterizing their relevant aspects. After a succinct historical survey on the understanding of organization in the organicist tradition, we offer a specific characterization in terms of closure of constraints. We then discuss some implications of the adoption of organization as a principle and, in particular, we focus on how it fosters an original approach to biological stability, as well as and its interplay with variation.
Keywords: Theoretical principle, organization, constraints, closure, stability, organicism
Bernard, 1865/1984, quoted and translated by Wolfe, 2010.
1 Introduction
For the past five decades, most of biological research has been framed on the hypothesis that biological organisms are essentially determined by genetic information[1], and the molecular mechanisms through which such information is expressed. This hypothesis – which we refer to here as genocentrism – acknowledges of course that a variety of causal factors (e.g. physical, environmental…) concur in enabling the development and functioning of biological organisms. Yet, among these factors, genetic ones would have a special status, insofar as they determine the distinctive features of biological phenomena. In particular, protein synthesis, (and thereby biological functions) results from the expression of genetic information. According to a genocentric perspective, therefore, what makes biological systems specific with respect to other natural systems is ultimately the fact that they would be the result of the expression of genetic information.
Understood in this way, genocentrism carries on a form of explanatory reductionism insofar as biological phenomena are assumed to be adequately explained[2] by appealing to genetic information. In particular, the concept of organism loses centrality in biological sciences (Laubichler, 2000) because of its supposed derivability from genes: organisms would be, under adequate conditions, the result of the expression of genetic information through development.
The research program framed on genocentrism has undergone a spectacular development, remarkably represented by the Human Genome Project, which was declared complete in 2003. Recently, however, experimental evidence is increasingly challenging the idea that genetic information determines biological functions: in particular, gene expression is subject to massive variability, which suggests that DNA underdetermines functional proteins and, in the end, the very organization of the organism. Far from being mere “noise”, variation is increasingly conceived as an inherent dimension of gene expression (Lestas et al., 2010; Dueck et al., 2016). Moreover, experimental biology shows not only that gene expression is variable, but even inherently stochastic (Ray & van Oudenaarden, 2008; Kupiec & Sonigo, 2000)[3].
As a matter of fact, the accumulation of experimental evidence at odds with genocentrism has induced a progressive renewal of interest in more integrative accounts, which aim at complementing genes with other determinants of biological phenomena. A main example of this trend is Systems Biology (Kitano, 2002) that elaborates mathematical and computational models on large, multi-scale molecular networks, whose dynamics cannot be determined by genetic information and which, in turn, control the activity of genetic templates.
In the search for integrative accounts, a specific theoretical option consists in claiming that the relevant level of description at which Biology should be framed is that of the organism: the alternative to genocentrism would therefore be organicism (Gilbert & Sarkar, 2000; Ruiz-Mirazo et al, 2000; Soto & Sonnenschein, 2005). From an organicist perspective, organisms are the main object of biological science because they are the systems that underlie biological phenomena and – crucially – they cannot be reduced to more fundamental biological entities (such as the genes or other inert components of the organism).
The elaboration of a theory of biological organisms requires dealing with their distinctive complexity, which in turn requires taking into account a number of dimensions, including individuation (see Clarke, 2011; Miquel, 2016), agency (Barandiaran et al., 2009; Arnellos & Moreno, 2015; Soto et al, 2016), regulation (Bich et al. 2015), adaptivity (Di Paolo, 2005), historicity (Ruiz-Mirazo & Moreno, 2012; Longo & Montévil, 2011; 2014), … and cognition (Thompson, 2007). In this paper and in Montévil et al. (2016), we take a theoretical step toward a Biology of Organisms by arguing that organisms are governed by two theoretical principles: organization and variation. All biological organisms, in all their diversity and richness of forms and kinds, meet two general principles without exceptions: they are organized, and their organization undergoes variation.
As theoretical principles, organization and variation constitute overarching hypotheses that frame the intelligibility of the objects within the biological domain. Taken together, they characterize the relevant aspects of biological objects, that are measurable observables, relations and changes. To better grasp their nature, a relevant comparison can be made with the role of space and time in Physics, ever since Newton and Kant. One may consider space and time as “conditions of possibility” for constructing physical knowledge; in more modern terms, positing a priori the phase space (i.e. the list of pertinent observables and parameters) allows us to spell out a complete determination of the intended processes in physical theories, by equations or evolution functions. Analogously, the ambitious aim of this work is to single-out the principles to be posited as a priori conceptual tools for the intelligibility of ontogenesis.
In the general discussion of Montévil et al. (2016), we further elaborate on the status of organization and variation as theoretical principles. One important implication of this strategy is that, although the two principles are supposed to lay the foundations of a biology of organisms, their domain of application is not necessarily restricted to the latter. Indeed, the set of systems that comply with the two principles – and can therefore be taken, by definition, as biological systems – is presumably larger than that of organisms. For instance, it has been recently argued (Nunes-Neto et al., 2014) that ecosystems might be described as organized systems by appealing to the same organization principle we are presenting herein. Accordingly, if they were shown to comply with both the organization and variation principles, ecosystems might be conceived of as biological systems, although not necessarily as organisms (Moreno & Mossio, 2015). In other words, we submit that biology is the science of systems meeting the principles of organization and variation, organisms being a specific, particularly relevant, class of biological systems. In the general discussion of Montévil et al. (2016), we further elaborate on the status of organization and variation as theoretical principles.
To characterize each principle, as well as their mutual relations, we elaborated in two distinct papers: the present one deals with organization, while Montévil et al. (2016) explores variation. Within our framework, the two principles are closely related, and each one is involved in the biological realization of the other. On the one hand, organization is a condition for variation, in the sense that the variation we focus on is that of the organization: relevant biological variation is that affecting organized systems and their parts. In addition, organization favors the propagation of variation because the mutual dependence between the parts enables the maintenance of changes. On the other hand, variation is a condition for the maintenance and adaptation of organisms over time, as well as the appearance of functional innovations. Biological organization would neither display its current complexity, nor would it last, unless it varied, both during phylogenesis and ontogenesis.
2 A primer on the relationship between organization and variation
The principle of organization focuses on the specific complexity of biological systems. Organization refers to the differentiation of functional roles (i.e. division of labor) among the parts of a system and, at the same time, to their integration and coordination as a whole. Furthermore, organization involves a generative dimension in the form of a mutual dependence, such that the very activity and existence of each organized part depends on its mutual relationship with the others. As we recall in section 3, these ideas have a long history in both biology and philosophy of biology. In section 4, we provide a characterization of the central features of the very notion of biological organization.
One central implication of proposing a theoretical characterization of biological organization is that this modifies the role of genes as determinants of biological systems. Genes are no longer supposed to be the fundamental causes of biological functions and complexity. Genes (or, more appropriately in our framework, DNA) are certainly constituents of biological organization, and they undoubtedly play an indispensable role in the development and functioning of biological systems, as templates for the synthesis of functional macromolecules. Moreover, DNA may be considered as a trace of history: without DNA, biological complexity would not exist. Yet, the expression of genes does not determine the organization, and organization can by no means be understood as its result. Rather, just as for any functional part, the expression of genes presupposes that the system is organized[4] (see also Moreno & Mossio, 2015, chapter 6).
As discussed in section 4, the realization of organization involves the conservation of relevant biological aspects, which in turn are associated with the maintenance or reestablishment of local and global theoretical symmetries. In this respect, as we argue in section 5.1, organization constitutes the fundamental ground of biological stability, both at the ontogenetic and phylogenetic scale. Biological organization tends to maintain itself and, thereby, to counter and remove potentially deleterious variations, while preserving useful variations.
At the same time, biological organization undergoes variation, our second theoretical principle. Based on continual symmetry changes (breaking and reconstruction), variation refers to various kinds of changes. Over time, the conserved aspects of organisms do vary, and these variations can be within the organization (some flows may change quantitatively) or of the organization itself (functions can change qualitatively). In Montévil et al. (2016), we discuss how the principle of variation enables us to frame biological objects as specific objects, endowed with historicity, contextuality and variability (see also Longo et al., 2015). In section 5.2, we discuss another aspect of the relationship between organization and variation, i.e. the fact that the former can, in the appropriate circumstances, favor and enhance the propagation of local variation to the whole system. Accordingly, section 5 as a whole emphasizes the twofold role of the principle of organization in enabling both stability (section 5.1) and variation (section 5.2): a conceptual tension and complementarity with which a theory of biological organisms should deal in depth in the future. In the conclusion, we sum up the main ideas of the paper, and open some future research directions as, in particular, the conceptual connection between the principle of organization and the principle of the biological default state proposed by Soto et al. (2016).
We next focus on the organization principle, by briefly addressing its history as it pertains to the biological domain.
3 Biological organization: a historical perspective
In the history of biology and philosophy of biology, the organicist tradition has advocated an understanding of biological systems as organized systems (Wolfe, 2010). As Gilbert and Sarkar (2000) explain, organicism constitutes a middle ground between reductionist perspectives and non-naturalist ones. The former assume that the whole can be reduced to its parts (for instance the genes), while the latter appeal to non-natural entities[5]. Others conceive organicism as a tradition that relies on both bottom-up and top-down structures of determination (Soto and Sonnenschein, 2005; Noble, 2006; Soto et al., 2008).
Two preliminary remarks are relevant here. First, until quite recently, organicism had not yet elaborated a coherent and integrated theoretical framework; rather, it has had a multifarious perspective, in which the very notion of organization has not been spelled out in precise theoretical terms. More recently, the situation has improved, and organization is being conceived in more specific terms. Our own proposal in this paper is directly reminiscent of, and grounded in, some of these recent developments.
Second, the organicist[6] tradition has been traced back to Aristotle’s conception of the teleological dependence of the parts to the whole organism (Aristotle, 2002) and, in more recent times, to Leibniz’s notion of ‘organic machines of nature’ (Fichant, 2003) that he opposed to Stahl’s animistic perspective in their famous controversy (Duchesneau, 1995). However, we will restrict the present overview to the last two centuries, in line with the view that the scientific study of biological organization was significantly oriented by Kant’s contribution (Lenoir, 1982).
3.1 From Kant to Weiss
In his Critique of Judgment (1790/1987), Kant explicitly describes biological systems as systems characterized by the specific way in which their parts are organized, by the distinctive relationship between the parts and the whole. Kant claims that, unlike any other kind of system, the parts of biological systems do not and cannot exist by themselves, but only insofar as they constitute an organized whole which, in turn, is itself a condition for their own existence and functioning[7]. Accordingly, biological systems are able to organize themselves – to self-organize – and can thereby be characterized as “natural purposes”, that is, as entities whose constituents are inherently subordinated to the whole organization.
The Kantian focus on biological organization had continuity in the (mostly Continental) Biology of the 19th century, notably in the work of Goethe (1995) and Cuvier (1817). Cuvier’s principle of the “condition of existence”, for instance, claims that “the different parts of each being must be coordinated in such a way as to render possible the existence of the being as a whole” (1817 i., 6, quoted and translated by Reiss, 2005). By implying that the different parts are linked and coordinated, Cuvier’s principle grounds and guides his empirical investigations in comparative anatomy and paleontology (Cuvier, 1805; see also Huneman, 2006, for an analysis).
Kant’s and Cuvier’s perspectives further influenced the so-called German “teleomechanists” (Lenoir, 1982) and in particular – to mention two of their prominent figures – Müller’s physiology (1837/1840) and von Baer’s embryology (1828). They both consider that, as Huneman writes “the proper object of life sciences should be a set of parts organizing itself as a whole, the development and the functioning of this specific kind of entity being the proper field of, respectively, embryology and physiology” (Huneman, 2010: 342).
Claude Bernard explicitly invokes Cuvier’s holistic view, and claims that biological systems are to be conceived as organized entities, whose parts are interdependent and mutually generative. In his words, “The physiologist and the physician must never forget that the living being comprises an organism and an individuality... If we decompose the living organism into its various parts, it is only for the sake of experimental analysis, not for them to be understood separately. Indeed, when we wish to ascribe to a physiological quality its value ad true significance, we must always refer to this whole and draw our final conclusions only in relation to its effects in the whole” (Bernard 1865/1984, II, ii, § 1, 137, quoted and translated by Wolfe, 2010). Bernard’s main focus is on the contribution of the organized parts - that must be investigated through the experimental method – to the conservation of the internal milieu, in spite of the continuous variations taking place in the external milieu[8].
An important moment in the history of the scientific treatment of biological organization is represented by the “Theoretical Biology Club”, founded in Cambridge by a group of researchers that included Woodger, Needham, Waddington and von Bertalanffy (Etxeberria & Umerez, 2006; Peterson, 2010). The Theoretical Biology Club promotes a scientific organicist perspective for biology, and undergoes a rigorous conceptual and theoretical treatment of various dimensions of the very idea of organization, including the analysis of internal relations (Woodger, 1929) and hierarchies (Needham, 1937). A particularly relevant contribution is due to von Bertalanffy (1952), who conceived biological systems as thermodynamically open systems. Biological systems are organized, and their organization goes along with thermodynamic openness, i.e. the fact that they continuously exchange energy and matter with the surroundings. Initially used by Bertalanffy as an argument against both vitalism (but see footnote 5) and mechanism, the thermodynamic openness of biological systems – as we will discuss –plays a crucial role in the subsequent elaborations of the notion of biological organization.
To complete this quick overview of pioneering approaches, it is worth recalling that the notion of organization has played a central role in the organicist perspective that permeated embryology in the first half of the 20th century. In particular, Paul Weiss refers to organization as the “coordinating principle” (Weiss, 1963: 190) that characterizes biological systems beyond local components and processes, and that grounds their stability in the face of internal or external perturbations (see Bich & Arnellos, 2013, for a discussion of Weiss’ ideas in relation to the organicist tradition). One of the central goals of this paper is precisely to focus on organization as a principle of stability, as foreseen by Weiss (see section 5.1 below).
3.2 From Piaget to Kauffman
In the second half of the 20th century, the conceptualization and scientific treatment of biological organization entered into a new phase, characterized by an increasing coherence and theoretical refinement. A milestone in this tradition is the account put forward by Jean Piaget (Piaget, 1967), whose core idea is to integrate into a single coherent picture two inherent dimensions of biological systems: thermodynamic openness and organizational closure. On the one hand, as emphasized by Bertalanffy, organisms are thermodynamically open (dissipative) systems, traversed by a continuous flow of matter and energy. On the other hand, they realize closure, i.e. a mutual dependence between a set of constituents which maintain each other through their interactions and which could not exist in isolation.
In Piaget’s view, closure captures a fundamental aspect of the very idea of “organization”, through the association between division of labor and mutual dependence that it implies. In other words, biological organisms are organized precisely because they realize closure. The centrality of closure and its connection to organization, as well as its distinction from (and, yet, complementarity to) thermodynamic openness, have become givens in most subsequent accounts of biological organization (Letelier et al., 2011).
One of the best known accounts of biological organization is the one centered on the concept of autopoiesis (Varela et al., 1974; Varela, 1979) which, among other aspects, places heavy emphasis on the generative dimension of closure: biological systems determine themselves in the sense that they “make themselves” (auto-poiein). Precisely because of their dissipative nature, the components of biological organisms undergo degradation over time; the whole system preserves its coherence and identity only insofar as it maintains and stabilizes not just some internal states or processes, but the autopoietic system itself as an organized unity. In spite of its qualities, however, we have argued elsewhere (Montévil & Mossio, 2015) that a central weakness of the concept of autopoiesis is that it does not provide a sufficiently explicit characterization of closure. Biological systems are at the same time thermodynamically open and organizationally closed, but no details are given regarding how the two dimensions are interrelated, what constituents are involved in closure, and at what level of description. In the absence of such specifications, it remains unclear in what precise sense closure would constitute a causal regime that distinctively characterizes biological organization[9].
A concerted attempt to answer this question has been made by Robert Rosen. In Life Itself (Rosen, 1991), Rosen reinterprets the Aristotelian categories of causality, and claims that the distinction between closure and openness should be grounded on a distinction between efficient cause and material cause[10]. By relying on this distinction, Rosen’s central thesis is that: “a material system is an organism [a living system] if, and only if, it is closed to efficient causation” (Rosen, 1991, p. 244). In turn, a natural system is closed to efficient causation if, and only if, all components having the status of efficient causes within the system are materially produced by the system itself. What matters here is that closure is located at the level of efficient causes: what constitutes the organization is the set of efficient causes subject to closure, and its maintenance (and stability) is the maintenance of the closed network of efficient causes.
Although Rosen’s account represents a clear step forward in the theoretical understanding of organization, we think that it still remains too abstract, and therefore hardly applicable as a guiding principle for biological theorizing, modeling and experimentation. Rosen defines closure as involving efficient causes but, without additional specifications, it might be difficult to identify efficient causes in the system: what entities actually play the role of efficient causes in a biological system? To deal with this issue, decisive insights have emerged from more recent literature that elaborates on the thermodynamic grounding of biological systems (Bickhard, 2000; Christensen and Hooker, 2000; Moreno & Ruiz-Mirazo, 1999) and the relations between closure and openness. In particular, Stuart Kauffman (Kauffman, 2000) argues[11] that biological organization implies a circular relationship between work and constraints, in the form of what he labels a “work-constraint (W-C) cycle”. When a (W-C) cycle is realized, constraints that apply to the system are produced and maintained by the system itself. Hence, the system needs to use the work generated by the constraints in order to generate those very constraints, by establishing a mutual relationship – a cycle – between constraints and work.
The understanding of the principle of organization that we put forward in this paper lies at the intersection between Rosen’s and Kauffman’s proposals, and elaborates on the idea of « self-construction » put forward by Kepa Ruiz-Mirazo and Alvaro Moreno in their analysis of basic autonomy (Ruiz-Mirazo and Moreno, 2004). In the following section, our central thesis is that closure should be specifically understood as closure of constraints, a regime of causation which is, at the same time, distinct from and inherently related to the underlying causal regime of thermodynamic openness.
4 Biological organization as closure of constraints
By relying and elaborating on the biological and philosophical tradition outlined in the previous section, we submit that biological organization is to be understood as a closure of constraints. In other words, claiming that biological systems are “organized” means, in a theoretical precise sense, that some of its constituents acting as constraints realize a regime of mutual dependence between them, which we label ‘closure’.
As mentioned above, the concept of closure relates to that of openness. Only thermodynamically open systems can possibly comply with the organization principle, although not any thermodynamically open system does. As all open systems, indeed, be they physical or chemical, biological systems are traversed by a flow of energy and matter, which takes the form of processes and reactions occurring in open thermodynamic conditions. In this respect, organisms do not qualitatively differ from other natural open systems. In turn, the characteristic feature of biological systems is that the thermodynamic flow is constrained and canalized by their constitutive constraints, which realize a specific form of mutual dependence – closure – between them.
The understanding of biological organization in terms of closure relies therefore on a distinction between processes (and reactions) and constraints exerted on the former. Let us then turn to this distinction.
4.1 Processes and constraints
In the complex dynamics taking place in biological organisms, different parts can be observed and distinguished. Parts are specific structures that play a role in controlling the dynamics, while remaining essentially unaltered by them. In the case of a mammal, an intuitive example is the vascular system, which while transporting blood is not altered by the blood flow. At a much lower level of description, another example is provided by enzymes that change the kinetics of a chemical reaction without being consumed. We propose to characterize the general notion of a biological part in terms of the more precise one of ‘constraint’ and, thereby, to ground the distinction between thermodynamic openness and organizational closure on a distinction between processes and constraints.
Broadly speaking, processes refer to all those transformations (typically physical processes, chemical reactions, etc.) that occur in biological systems and involve the alteration, consumption, production and/or constitution of entities. Constraints, in turn, refer to entities that, while acting upon these processes, can be said (in some appropriate sense) to remain unaffected by them. A variety of entities can play the role of constraints in an organism, be it in the form of boundary conditions, parameters, restrictions on the configuration space, etc.… In some cases, constraints are exerted by external physical forces and fields, which are essential for life as we know it: for instance, gravitation canalizes development (Bizzarri et al., 2015). In other cases (which, as mentioned, are of paramount importance in the biological domain), constraints are exerted by specific material structures within the organism.
In all situations, constraints contribute to determining the behavior of the system (be it physical, chemical or biological), by reducing the degrees of freedom of the processes and dynamics on which they act.
We suggest defining constraints as entities that exhibit a symmetry with respect to a process (or a set of processes) that they help stabilize. In general terms, a symmetry is a transformation that does not change the relevant aspects of an object: symmetries and conservation (of energy, momentum, electrical charges, etc.) are therefore complementary concepts (van Fraassen, 1989). Applied to the notion of constraint, this means defining constraints as entities that can exert a causal influence because of some symmetrical (conserved) aspect with regards to the target process.
More precisely, given a process A → B (A becomes B), C is a constraint on A → B, at a specific time scale τ, if and only if two conditions are fulfilled:
I/ C exerts a causal role on the target process. In formal terms, we express this by stating that the processes A → B and AC → BC (i.e. A → B under the influence of C) are asymmetric (different) at a time scale τ[12].
II/ C is not altered by (i.e. is conserved through) the target process, at the scale at which the latter takes place. More formally: a temporal symmetry (a conservation property) is associated with all aspects of CA → B with respect to the process AC → BC , at time scale τ.
The situation which fulfills conditions I-II will be expressed as C(A → B)τ or, in a graphical form, as:
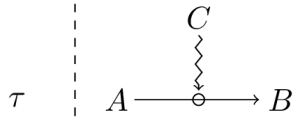
Let us go back the two biological examples of constraints mentioned above, the vascular system and an enzyme in a mammal, and show that they meet both conditions.
Consider first the vascular system. On the one hand, there is a difference between the flow of oxygen under the influence of the vascular system ( AC → BC ) or in its absence (A → B) since, for instance, AC → BC occurs as a transport of oxygen canalized to the neighborhood of every cell where diffusion will occur, whereas A → B would only have a diffusive form. Consequently, the situation AC → BC fulfills condition I, with the vascular system playing a causal role in the flow of oxygen. On the other hand, a temporal symmetry is associated with the vascular system C with respect to the transformation AC → BC since, among other things, the spatial structure of the vascular system is conserved at the time scale required to accomplish the transport of oxygen molecules from the lungs to the cells. Hence, the situation fulfills conditions II, which means that the relevant aspects CA → B (here, the spatial structure) are conserved during the process of oxygen transport.
Consider now an enzyme. There is an asymmetry between a chemical reaction when considered under the influence of an enzyme ( AC → BC ) and when not (A → B) since, typically, AC → BC occurs faster than A → B. Similarly, a temporal symmetry is associated with the configuration of an enzyme, which is conserved during the reaction while reactants do not. Note that at time scales shorter than τ, an enzyme does undergo alterations insofar as it binds to the substrate. The symmetry is respected only by considering the whole process at τ, when the enzyme unbinds and returns to its initial configuration.
Since they meet the two conditions, both the vascular system (with respect to oxygen transport) and enzymes (with respect to chemical reactions) act as constraints within the organism.
A crucial remark is that each condition is met only at the relevant time scale and, in particular, that the time scale τ at which conditions I and II must be fulfilled is the same. A constraint, to be such, must conserve its relevant aspects at the same time scale at which its causal action is exerted, even though changes and alterations may occur at shorter and/or longer time scales. Indeed, it is precisely because of their conservation that constraints are able to exert their causal power. Consider again our two examples. The structure of the organism’s vasculature does not change at those time scales at which it channels the flow of oxygen; yet, the structure of the vasculature does change at longer time scales due to the effects, for example, of neovascularization. The same holds true for enzymes, which are conserved at the time scale of catalysis, while decaying and randomly disintegrating at longer scales. Moreover, as mentioned above, enzymes also undergo alterations at shorter time scales (since they bind with the substrate and loose or gain electrons, protons, etc.) and are then restored when catalysis is achieved.
More generally, a given entity cannot be qualified as a constraint in abstracto, insofar as its conserved aspects (and their causal powers) can only be assessed in relation to a specific process and the relevant time scale at which it occurs. This context- and scale-dependence are, in our view, general features of constraints. For example, a protein may be used differently in different biological contexts: crystallins, the structural proteins that confer transparency to the vertebrate lens, also act as enzymes when expressed in other organs (Rao et al., 1992).
The central outcome of the theoretical distinction between constraints and processes is a distinction between – to use a philosophical jargon – two regimes of causation. For a given effect B of a process or reaction, one can theoretically distinguish, at the relevant time scale, between two causes (or, as Rosen put it, two answers to the question “why B?”): the inputs or reactants A that are altered and consumed through the process, and the constraints C, which are conserved through that very process. Constraints constitute a distinct kind of causes insofar as they are not reduced to the thermodynamic flow, and to the material inputs or reactants.
4.2 Dependence and closure
In Physics and Chemistry, constraints are usually introduced as independent determinations whose existence and maintenance does not depend on the dynamics on which they act. The classical example of the inclined plane illustrates this situation well: the inclined plane acts as a constraint on the process (the sliding or rolling of an object on it), whereas the constrained process does not exert a causal role in generating and maintaining the plane itself.
In a fundamental sense, we submit that biology as a science is about those circumstances in which the constrained process does play a role in determining the conditions of existence of (some of) the constraints exerted on them. More specifically, there are situations in which the existence of a set of constraints collectively depends on the actions that they exert on the processes and dynamics. When this occurs, the set of mutually dependent constraints can be said to realize closure and therefore to be organized.
We can now characterize the principle of organization more precisely:
The principle of organization states that biological systems realize a closure of constraints.
The organization of constraints realizing closure achieves a form of “self-determination”, in the precise sense that the conditions of existence of the constraints subject to closure (that we label “constitutive”, see footnote 21 below) are determined within the organization itself. Before discussing closure as such, let us first have a look to the idea of the “dependence” between constraints.
As discussed above, constraints are defined as entities that, at the relevant time scale, exhibit conservation (a symmetry) with respect to the process on which they act. Yet, constraints are also subject to degradation at longer time scales, and must be replaced, repaired or maintained. For instance, the cells that constitute the vascular system must be nourished, and enzymes undergo degradation over time, and must be replaced. When the maintenance of a constitutive constraint depends (also) on the action of another constraint, a relationship of dependence is established between the two.
A bit more formally, let us consider a constrained process C1(A1 → B1) τ1. Because of condition II, there is a time symmetry at scale τ1 associated with C1, which concerns those aspects that are relevant for the constrained process. At the same time C1 is, by hypothesis, the product of another constrained process C2(A2 → C1)τ2, at a different time scale. At scale τ2, C2 plays the role of constraint, whereas C1 does not, since it is the product of the process C2(A2 → C1). Schematically:
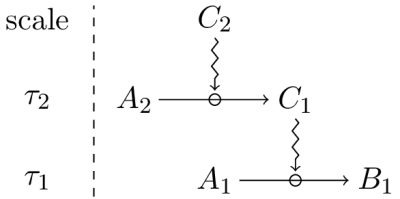
This situation establishes a dependence relationship between constraints in which constraint C1 depends on constraint C2. In this situation, we say that C1 is dependent on C2, and that C2 is generative for C1.
In organisms, the dependence between constraints is ubiquitous. As an example, let us consider the production of an enzyme. As discussed above, an enzyme acts as a constraint on the reaction it catalyzes. In turn, enzymes are themselves produced by and within the cell, through the transcription and translation processes: messenger RNA is synthesized, ribosomes build the primary sequence of the future protein on the basis of the messenger RNA sequence, without consuming it. Since the ribosomes and the mRNA play a causal role while being conserved during these processes, they both act as constraints (at specific time scales) on the production of the enzyme. Consequently, the relationship between the enzyme, the ribosomes and the mRNA can be pertinently described as dependence between constraints (in which the enzyme depends on both ribosomes and mRNA). Of course, other constraints are involved in the process of producing a functional protein, for example, alternative splicing of RNA, post-translational modifications and folding
At a different level of description, another example of dependence is that between the vascular system and other systems or organs in the body, for instance the gut. The vascular system constrains the transport of nutrients (for instance amino-acids and oxygen) to the cells, while being conserved at the relevant time scales. In turn, the epithelial cells lining the mucosa of the small intestine constrain the transport of nutrients into the blood, while being conserved at specific time scales (the life time of these cells spans a few days). The relationship between the vascular system and the gut is dependence between constraints[13].
In a general sense, dependence between constraints underlies any “repair mechanisms” at work in the organism: in addition to the wide-ranging literature on DNA repair (Friedberg et al., 1995), this also includes the repair or, better, reconstruction[14] of all kinds of parts of an organism (Wang et al., 2009; Bergamini, 2006). Reconstruction requires the existence of a part (C1) that is conserved while the main process occurs (i.e. its alteration is negligible at the relevant scale, τ1), even though it may be altered in the long run (τ2). The maintenance of the system’s organization, on the other hand, requires, at time scale τ2, the existence of a second subsystem (C2) in charge of maintaining C1 through the adequate canalization of a process (or a set of processes) A2 → C1.
Let us now turn to closure, which we interpret as a specific case of mutual dependence between constraints. In the natural world, constraints may (and usually do) depend on other constraints, so that “chains” of dependences can be described. The specificity of biological systems consists of the fact that such chains of dependences realize complex networks of mutual dependences, usually at various levels of description. To express the idea more formally, we argue that a set of constraints C realizes closure if, for each constraint Ci belonging to C:
1. Ci depends directly[15] on at least one other constraint belonging to C ( Ci is dependent);
2. There is at least one other constraint Cj belonging to C which depends on Ci ( Ci is generative)[16].
As an abstract illustration of closure, consider the following network of dependent constraints:
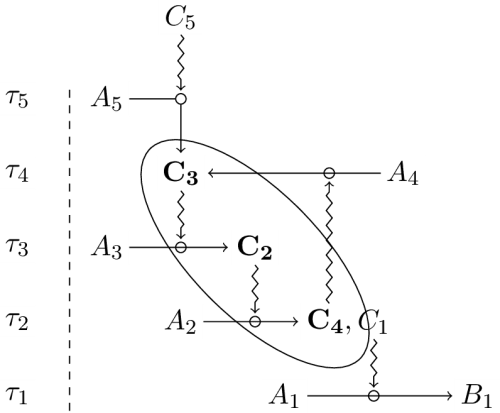
In this diagram, C1, C2, C3, C4 and C5 satisfy, ex hypothesi, the definition of constraint at τ1, τ2, τ3, τ4 and τ5 respectively. Furthermore, C1, C2, C3 and C4 play the role of dependent constraints, while C2, C3, C4 and C5 are generative constraints. The subset of constraints which are both generating and dependent is then (C2, C3, C4): this subset realizes closure, and is therefore organized.
The following illustration provides a more biologically oriented example. It represents in a highly simplified way the mutual dependence between the vascular system and the small intestine of a mammal, included in the overall closure of the organism. Both the vascular system and the small intestine act as constraints on the flow of nutrients. They realize closure by jointly contributing to maintaining their own cells. In particular, while the small intestine constrains the breaking down of food and the absorption of nutrients, the vascular system constrains their transport to all cells, including their own. Each individual cell of the small intestine, in addition to secreting enzymes that contribute to breaking down the food, realizes organizational closure itself, as any cell in the organism.
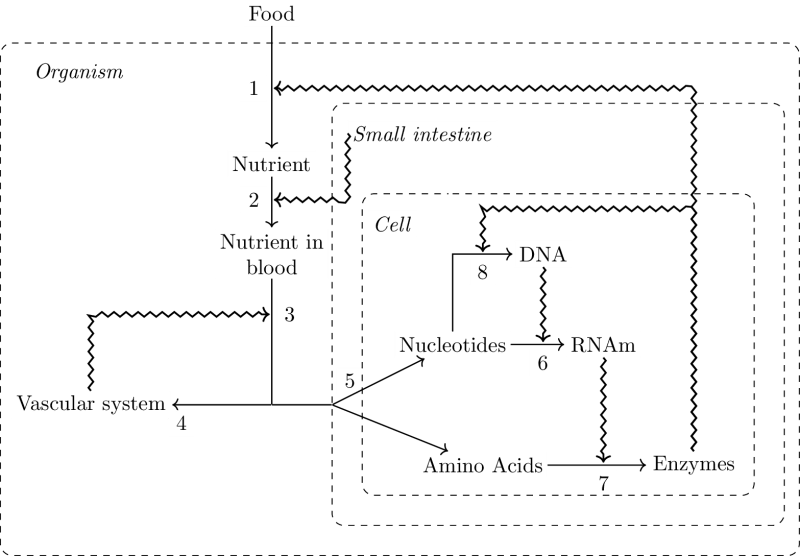
It is important to underline that this schematic illustration is by no means supposed to provide a model of closure, which would adequately capture the complexity of real biological systems. In particular, it represents the relations between two structures while most of other organs are not included. Rather, its aim is to express in a clear form some structural features of the principle of organization; the principle, in turn, should guide the further development of models of biological organization. Yet, some important implications can already be derived from this preliminary characterization.
First, we claim that constraints subject to closure define biological functions (Mossio et al., 2009; Saborido et al., 2011). Within this framework, performing a function means exerting a constraint on a target process or reaction. All kinds of biological structures and traits to which functions are usually ascribed satisfy the definition of constraint given above, albeit at various different temporal and spatial scales. In addition to the vascular system and enzymes, some intuitive examples include membrane pumps and channels (which constrain both the inward and outward flow of materials through the membrane) as well as organs (such as the heart which constrains the transformation of chemical energy into blood movement). The principle of organization grounds functionality within biological systems: constraints do not exert functions when taken in isolation, but only insofar as they are subject to closure.
Second, closure should be clearly distinguished from independence, insofar as a system that realizes closure is a physically open system, inherently coupled to the environment with which it exchanges energy and matter (Nicolis and Prigogine, 1977). This implies that closure is a context-dependent determination, to the extent that it is always realized with respect to a set of specific boundary conditions, which includes several external (and independent) constraints acting on the system (such as, for instance, constraint C5 in the abstract diagram above). Consequently, closure does not and should not include all the constraints with which the system may have a causal interaction, but rather only the subset of those that fulfill the requirements stated above[17].
Third, the principle of organization makes closure a general aspect of biological organisms that is constantly conserved during their lifespan. As we discuss and develop in Montévil et al. (2016), biological systems continuously undergo changes that may also result in the acquisition or loss of constitutive constraints and related functions; yet, whatever change must generate a network of dependencies that preserves closure. Different biological organisms realize different forms of closure, and even the same organism continuously modifies its own organization through time; but in all situations the very fact of realizing closure is conserved. We refer to this situation as the non-identical iteration of morphogenetic processes (Montévil et al., 2016; Longo et al., 2015), which refers to the dynamics of organs and, when these dynamics result in a functional change, to the overall organization.
5 Bringing variation into the picture
One central implication of adopting organization as a theoretical principle for biology concerns the understanding of the interplay between the stability and variation of biological phenomena.
On the one hand, we submit that the closure of constraints underlies the stability of biological systems (both at the individual and evolutionary scale), and determines the maintenance of their constitutive dynamics over time. On the other hand, organizational closure undergoes variation, as we argue at length in Montévil et al. (2016). One aspect on which we focus here is that closure, in the relevant situations, favors and enhances functional variation, i.e. variation of the organization itself. Next, we discuss both aspects in some details.
5.1 Organization grounds stability
One of the most astonishing features of biological organisms is the stability (i.e. their maintenance through time[18]) that they exhibit, both at the individual and cross-generational scale, in spite of the huge complexity and delicate equilibrium of their constitutive dynamics.
What does explains biological stability? As in any other natural system, biological systems are subject to physicochemical factors, whose (stable) influence can contribute to explain the (stable) occurrence of some biological patterns. The focus on physical determinants is quite old in biology, D’Arcy Thompson’s On Growth and Form (1917) being one of the most famous illustrations. In recent years, more precise work has been done in this field, for instance about the role of gravity on cell and tissue organization (Bizzarri et al., 2015). Another example is the analysis of the morphogenesis of the gut as a buckling process (Shyer et al., 2013).
Yet, physical factors apply to any kind of system, and are not usually considered sufficient to account for the stability of biological systems. It seems necessary therefore to appeal to some specifically biological sources of stability, one of them of course being DNA. An advocate of what we dubbed “genocentrism” in the introduction could claim that organisms are stable because, in addition to generic physical determinants, their behavior would be specifically controlled by the expression of the information contained in DNA, which is a highly stable molecule. In short, the stability of the DNA molecule would account for the stability of biological organisms.
The adoption of organization as a biological principle implies a significant departure from this view. Biological stability is due to the mutual interactions among constraints subject to closure: biological dynamics are stable because the organization of constraints is stable both at the individual and inter-generational scales. It is worth emphasizing that from the organizational perspective, DNA (interpreted as a constraint) of course plays a crucial role in determining stability. DNA is a fundamental physicochemical trace of a history, continually used by organisms as a template for the production of proteins. Yet, this role cannot be dissociated from the mutual dependences between DNA and the whole set of constraints subject to organizational closure. Taken in isolation, indeed, DNA is totally inactive and undergoes degradation in a relatively short characteristic time (half-life estimated to 521 years, see Morten et al., 2012); moreover, its alterations, such as mutations, may be induced by ordinary metabolic activities and are present at each cellular replication. As an organized constraint, in turn, its structure is reconstructed over very long time scales as a component of organisms. The relative stability of DNA structure both during ontogenesis and phylogenesis in the long run is therefore a consequence of the biological activity of the whole organization[19].
The understanding of organization as the fundamental source of biological stability should distinguish between two dimensions, a local and a global one. In both cases, organization grounds stability through the relevant symmetries that it displays (of constraints and closure respectively), and the related conserved aspects.
On the one hand, each functional constraint subject to closure exerts a control over target processes and reactions. For instance, consider the sodium-potassium pumps in the cell membrane. The “pump” is a Na+/K+ -ATPase enzyme, which enables the active transport of sodium ions to the outside and of potassium ions to the cytoplasm. Both Na+ outward flow and K+ inward flow occur against the gradient, which means that they would not occur without the constraint exerted by the pump. As a result, the pump contributes to maintain the adequate concentrations of ions within the cell and, among other things, avoid water flows in by osmosis, which could possibly lead to cytolysis (“osmotic burst”). In this case, the stability of these specific inward and outward flows, which in turn results in the overall stability of ions concentrations, is due to the action of “local” constraints, which operate at specific temporal and spatial scales. Local constraints determine the behavior of processes and reactions, and avoid undergoing deleterious variation, which would undermine the overall functioning of the organism. In the case of the sodium-potassium pumps, for instance, the constraints (the pumps) avoid deleterious (i.e. too large) fluctuations of the internal concentration of sodium.
On the other hand, the conservation of each constraint holds, as mentioned, only at a given time scale τ, which means that, at longer time scales, they must be regenerated or repaired. If it were not the case, their role in stabilizing processes and reactions would be altered, and would eventually cease. Now, because of closure, the maintenance of each constraint is (among other things) dependent on the activity of other organized constraints. The sodium-potassium pumps, as well as the whole membrane, are maintained by a number of functions exerted in the cell cytoplasm. In particular, the pumps are produced and replaced in the same manner than other proteins, thanks to the constraints exerted by DNA and mRNA mentioned above (see also figure [fig4]). Accordingly, the maintenance of each constitutive constraint beyond their characteristic time scale τ depends on the stability of the organizational closure.
As a fundamental biological property, organizational closure grounds the stability of functional constraints, by exerting a control over the variations that they undergo. Organization enables the maintenance of constitutive constraints, beyond their characteristic time scales, through the continuous re-establishment of their mutual dependences. In this respect, one might describe the overall stability of closure as the result of a kind of “organizational inertia”. Because of the network of mutual dependencies, biological organization tends to remove variations affecting local constraints and to regenerate them in a fundamentally unaltered form. Such a tendency towards what we might call conservative stabilization would occur in those cases in which local variation does not affect the constraints in charge of generating the one (or set) being affected. Indeed, because of closure, generative constraints are themselves – directly or indirectly – dependent on the constraint undergoing variation. Conservative stability supposes therefore that those constraints in charge of re-establishing the dependent constraint are not themselves altered by the variation that they might remove. For example, variations may occur during the synthesis of enzymes, typically as “errors” in transcription or translation. Yet, if these variations do not alter the final outcome (the function), they are leveled by the organization and not sustained over time. In the following subsection, we will examine the role of closure for enhancing variation when this condition is not met.
The pivotal role of organization in maintaining biological stability should not be restricted to the functioning of an adult organism, or to a single individual. Indeed, it can be argued that organization grounds equally cross-generational stability, by playing for instance a crucial role in inheritance and developmental processes, understood as the set of “processes that explain this reliable reoccurrence of features within lineages” (Mameli, 2005). In recent years, in particular, an increasing quantity of experimental data has put strong emphasis on non-genetic inheritance, which includes epigenetic, ecological, behavioral and cultural processes (Bonduriansky, 2012). Non-genetic inheritance suggests that the stability of biological patterns through generations cannot be adequately explained by appealing uniquely to genetic factors. Rather, biological inheritance could be the result of the interplay between a set of mutually dependent factors of different kinds, understood as constraints realizing an “extended” organizational closure (Pontarotti, 2015).
A detailed account of how the principle of organization underlies biological stability would go far beyond the scope and limits of this paper. What matters most for our present purposes is to put an emphasis on the general hypothesis that organization grounds stability of biological phenomena[20], which constitutes a substantial shift in focus from other approaches centered on genetic determinants or physical factors (or both of them). Organization controls the dynamics of the organism, and prevents deleterious variations that would threaten its very existence. Accordingly, there is an important sense in which organization, by grounding stability, counters, canalizes and uses variation.
Yet, the control exerted by the organization on variation is only one aspect of the intricate relations between the two theoretical principles. Next, we will consider how organization may enhance variation.
5.2 Organization propagates variation
The principle of variation that we propose for Biology– alongside organization – has fundamental implications. By complying with it, organisms undergo cascades of changes, which contribute to their specificity and, ultimately, to their historicity and contextuality (see also Longo & Montévil, 2014, and Longo et al., 2015). This leads to a central distinction between biological and physical objects the latter being, just as mathematical objects, generic and understandable in an ahistorical manner (see also Longo & Soto, 2016).
Biological systems undergo variation that can alter one or more constraints, or even their whole organization. As a result, the stability achieved by an organism is not just conservative but also, over individual and evolutionary time, cumulative, insofar as it keeps the track of functional innovations, and enables their preservation through time (see Montévil et al., 2016). Yet, the principle of organization does not merely ground stability, be it conservative or cumulative. Indeed, organization also (and somehow paradoxically) favors the propagation of functional innovation, and hence the increase of biological complexity.
What is the idea behind these claims? Broadly speaking, any system (be it a rock, a flame or a table) can undergo variation of a subset of its constitutive elements. A local variation can have different consequences on the global structure of the system, depending on the nature of the latter. In the case of a rock, a local fissure can result in the loss of a fragment, and in the modification of the global shape. In turn, a variation of some components of the flame (for example a small perturbation of its shape, or of the supply in combustibles), provided that it is compatible with the thermodynamic open state, does not affect at all the global behavior: the flame will keep behaving in the same way in spite of various possible modifications of its components. Lastly, a breakdown of one of the legs of the table might result in an alteration, or even extinction, of the global function of the table. A local variation can therefore induce various kinds of consequences in the global system’s configuration or functioning. Yet, in all these cases a local variation cannot induce other local variations, which would result in a global reconfiguration of the system. Either the variation remains local, or it might result in the breakdown/disintegration of the entire system; but no global variation results from local variation.
In turn, one of the specific features of systems meeting the organization principle is the fact that local (functional) variation might induce global stable reorganizations. In the previous section, we mentioned that organizational closure may tend to remove a variation occurring to a local constraint in those cases in which such variation does not feed back into the generative constraints. But, of course, there is another possibility, in which the feedback does occur. In such a case, a local variation can possibly affect other constraints, their properties, and their activity, which in turn could affect other constraints, and so on. When a local variation affects an organized system, the variation can propagate through the various functional constraints and two outcomes are possible. Either the resulting system cannot realize closure anymore, in which case it is not viable and disintegrates. Or, it does realize a new closure through cumulative stability, in which case the functional innovations are integrated into the organization, and preserved. The propagation of variation through closure is our way of understanding Darwin’s principle of “correlated variations”[21]. In this way, the organized system can explore what Kauffman (2000) calls “the adjacent possible” in the wide space of functional constraints. More precisely, a given organization does not entail such a change but it enables it, see (Longo et al., 2012b). In this respect, the generation of structural and organizational innovations constitutes a specifically biological form of randomness, leading to unpredictable organizational changes (Montévil et al., 2016).
The exploration of functional innovations and organizational variants, favored and enhanced by closure, may lead, in some circumstances, to the generation of increasingly complex structures, which could act as new constraints, generating more sophisticated and accurate functions. In brief, this process may lead to the increase of biological complexity, roughly conceived here as the degree of functional variety of an organized system.
6 Conclusions
We have claimed that the elaboration of a sound theory of biological organisms should adopt organization as a theoretical principle.
By elaborating on the long organicist tradition, we have put forward a specific understanding of the notion of organization, expressed in terms of closure of constraints. By relying on Montévil & Mossio (2015), we have proposed a diagrammatic description of closure, which provides a structured understanding of the principle. In this framework, biological organization refers to the mutual dependence (closure) between constraints, exerted on processes occurring in open thermodynamic conditions. Constraints are described as local symmetries, aspects that are conserved at the relevant time scale. Closure, in turn, is a global biological property, an overall determination that is conserved through ontogenetic and phylogenetic times.
We have mentioned some relevant implications deriving from the application of the principle of organization. In particular, we have discussed in some detail how it grounds biological stability and its interplay with variation, in an original (although complementary) way with respect to theoretical frameworks more centered on genes and/or physical factors.
Organization plays a twofold role with regard to stability and variation. In some conditions, closure can remove, integrate or average out variations and tends to conserve the ongoing network of mutual dependencies between functional constraints. In other conditions, the very same closure may promote the propagation of local variations to the whole organism: when this occurs, the resulting regime must realize a new stable organization, while integrating and preserving the functional innovations. Both situations are continually encountered in an organism, be it a unicellular or a multicellular one, since each metabolic cycle and each cell reproduction, in a metazoan, is a locus of possible change.
In this respect, a fundamental connection seems to exist between the principle of organization and the idea of the biological “default state” proposed by Soto et al. (2016). According to this idea, cells – both those that live autonomously as unicellular organisms and those that form part of multicellular ones – are by default in a state of proliferation with variation and motility. As a consequence, as they put it, “proliferation, variation and motility, require no explanation in biology. On the contrary, hindrances to the expression of default state, namely, proliferative quiescence, lack of variation, and lack of movement require an explanation” (Longo et al., 2015).
There are two important ways in which the principle of organization and the idea of the default state can be theoretically connected. On the one hand, it could be argued that proliferation with variation and motility are the default state of organized systems. Accordingly, the default state enriches organization by making explicit some of its most biologically relevant features and, reciprocally, organization grounds the default state by specifying the relevant class of natural systems to which it applies. On the other hand, the constraints constituting the organization certainly play a central role in explaining the observed departures from the default state. Under the effect of organized constraints, exerted both within their individual organization and by the multicellular organization of which they can be a part, cells can exhibit different degrees in their capacity to proliferate with variation and move, up to the extreme cases of proliferative quiescence, lack of variation or lack of movement. In a word, organized constraints control the default state, and the default state helps in the understanding of the nature of biological functions[22]. These theoretical considerations were used to model organogenesis on the bases of the default state and organizational closure (see Montévil et al, 2016).
The complex relations between stability and variation from an organizational perspective, outlined in this paper, should be investigated both theoretically and experimentally by a developing theory of organisms. Among other issues, a central one is certainly that regarding the description of different levels of organization, as well as their reciprocal relationship. Indeed, organized systems are not only typically constituted by a hierarchy of levels but, in addition, entities located at different levels interact with each other. A sound account of biological stability and variation should integrate these interactions in the picture.
Acknowledgements
We would like to warmly thank Ana Soto, Carlos Sonnenschein, Cheryl Schaeberle, Paul-Antoine Miquel, Charles Wolfe and Leonardo Bich for their careful reading and useful remarks on previous versions of this paper. This work was conducted as part of the research project “Addressing biological organization in the post-genomic era” which is supported by the International Blaise Pascal Chairs, Region Ile de France. Maël Montévil was supported by Labex "Who am I?", Laboratory of Excellence No. ANR-11-LABX-0071.
References
- Arnellos, A. & Moreno, A. (2015). Multicellular agency: an organizational view, Biology & Philosophy, 30, 3, 333-357.
- Aristotle (2002). On the Parts of Animals I-IV (Clarendon Aristotle Series). Translated by James Lennox. Oxford: Oxford University Press.
- Ashby, W.R. (1956). An Introduction to Cybernetics, vol. 2. London: Chapman & Hall.
- Atkins, P.W. (1984). The Second Law. New York : Freeman.
- Baer K.E. von (1828). Über Entwickelungsgeschichte der Thiere: Beobachtung und Reflexion, Königsberg: Borntrãger.
- Barandiaran, X., Di Paolo, E., & Rohde, M. (2009). Defining agency. Individuality, normativity, asymmetry and spatio-temporality in action. Journal of Adaptive Behavior, 17, 5, 367-386.
- Bergamini, E., (2006). Autophagy: a cell repair mechanism that retards ageing and age-associated diseases and can be intensified pharmacologically. Mol.Aspects Med. 27, 5-6, 403-410.
- Bernard, C. (1865/1984). Introduction á l’étude de la médecine expérimentale. Paris: Baillière.
- Bertalanffy L. von (1952). Problems of Life: An Evaluation of Modern Biological Thought. London: Watts & Co.
- Bich, L., Moreno, M., Mossio, M., & Ruiz-Mirazo, K. (2015). Biological regulation: controlling the system from within, Biology & Philosophy, http://link.springer.com/article/10.1007/s10539-015-9497-8
- Bich, L. & Arnellos, A. (2013). Autopoiesis, Autonomy and Organizational Biology: Critical Remarks on “Life After Ashby”. Cybernetics and Human Knowing, 19, 4, 75-103.
- Bickhard, M.H. (2000). Autonomy, function, and representation. Communication and Cognition Artificial Intelligence, 17, 3-4, 111-131.
- Bizzarri, M., Monici, M. van Loon, J.J.W.A. (2015). How Microgravity Affects the Biology of Living Systems. BioMed Research International, doi: 10.1155/2015/863075
- Bonduriansky, B. (2012). Rethinking heredity, again. Trans. in ecology, 27, 6, 330-336.
- Cannon, W. B. (1929). Organisation for physiological homeostasis. Physiological Reviews, 9, 3, 399-431.
- Christensen, W. D., & Hooker, C. A. (2000). An interactivist-constructivist approach to intelligence: self-directed anticipative learning. Philosophical Psychology, 13, 5-45.
- Cimino, G. Duchesneau, F. (Eds.) (1997). Vitalisms: from Haller to the cell theory. Florence: Leo Olschki.
- Clarke, E. (2011). The problem of biological individuality. Biological Theory, 5, 4, 312–325.
- Cuvier, G. (1805). Leçons d’anatomie comparée. Paris: Baudoin.
- Cuvier, G. (1817). Le règne animal distribué d’après son organisation, pour servir de base à l’histoire naturelle des animaux et d’introduction à l’anatomie comparée. Paris: Déterville.
- Darwin, C. (1909-14). The Origin of Species. Chapter V: The laws of Variation. Harvard Classics Edition.
- Di Paolo, E. (2005). Autopoiesis, adaptivity, teleology, agency. Phenomenology and the Cognitive Sciences, 4(4), 429–452.
- Duchesneau, F. (1995). Leibniz et Stahl: divergences sur le concept d’organisme. Studia leibnitiana 27: 185-212.
- Dueck, H., Eberwine, J., & Kim, J. (2016). Variation is function: Are single cell differences functionally important? BioEssays, 38, 172–180. doi:10.1002/bies.201500124
- Etxeberria, A., Umerez, J. (2006). Organización y organismo en la Biología Teórica ¿Vuelta al organicismo? Ludus Vitalis, 26, 3–38.
- Fichant, M. (2003). Leibniz et les machines de la nature. Studia leibnitiana 35.1: 1–28.
- Friedberg, E.C., Walker, G.C., Siede, W. (1995). DNA repair and mutagenesis. Washington, D.C: ASM Press.
- Gilbert, S. F., & Sarkar, S. (2000). Embracing complexity: organicism for the 21st century. Developmental Dynamics, 219, 1, 1-9.
- Goethe, J.W. (1995). Collected works, XII. Scientific studies (D. Miller, Trans., & Ed.). Princeton, NJ: Princeton University Press.
- Huneman, P. (2006). Naturalizing purpose: from comparative anatomy to the “adventures of reason”. Studies in History and Philosophy of Life Sciences, 37, 4, 621-656.
- Huneman, P. (2010). Assessing the Prospects for a Return of Organisms in Evolutionary Biology. Hist. Phil. Life Sci., 32, 341-372.
- Kant, I. (1790/1987). Critique of Judgment. Indianapolis: Hackett Publishing.
- Kauffman, S. (2000). Investigations. Oxford: Oxford University Press.
- Kitano, H. (2002). Computational systems biology. Nature, 420, 206–210.
- Kumada, Y., Benson, D.R., Hillemann, D., Hosted, T.J., Rochefort, T.J., Thompson, C.J., Wohlleben, W., Tateno, Y. (1993). Evolution of the glutamine synthetase gene, one of the oldest existing and functioning genes, PNAS, 90, 7, 3009-3013.
- Kupiec, J.J., Sonigo, P. (2000). Ni Dieu ni gene. Pour une autre théorie de l’hérédité. Paris: Seuil.
- Laubichler, M. (2000). The Organism is dead. Long live the organism! Perspectives on Science, 8, 3, 286-315.
- Lenoir, T. (1982). The Strategy of Life. Chicago: The University of Chicago Press.
- Lestas, I., Vinnicombe, G., & Paulsson, J. (2010). Fundamental limits on the suppression of molecular fluctuations. Nature, 467, 174-178.
- Letelier J.C., Cárdenas M., Cornish-Bowden A. (2011). From L’Homme Machine to Metabolic Closure: Steps Towards Understanding Life. Journal of Theoretical Biology, 286, 1, 100-113.
- Longo, G., Miquel, P.A., Sonnenschein, C., Soto, A. (2012a). Is Information a proper observable for biological organization? Progress in Biophysics and Molecular Biology, 109, 3, 108-114.
- G. Longo and M. Montévil. 2011. “From physics to biology by extending criticality and symmetry breakings.” Systems Biology and Cancer, Progress in Biophysics and Molecular Biology 106 (2): 340–347.issn: 0079-6107. doi: 10.1016/j.pbiomolbio.2011.03.005
- Longo, G. Montévil, M., Kauffman, S. (2012b). No entailing laws, but enablement in the evolution of the biosphere. Companion Volume of the 14th Genetic and Evolutionary Computation Conference (GECCO), 1379–1391.
- Longo, G., Montévil, M. (2014). Perspectives on organisms: biological time, symmetries and singularities. Heidelberg: Springer.
- Longo G., Montévil M., Sonnenschein C., Soto A. (2015). In Search of Principles for a Theory of Organisms. Journal of Biosciences, 40, 5, 955-68
- Longo, G. & Soto, A.M., Why do we need theories? (2016, this issue). Progress in Biophysics and Molecular Biology, Available online 4 July 2016, ISSN 0079-6107, doi: 10.1016/j.pbiomolbio.2016.06.005
- Mameli, M. (2005). The inheritance of features, Biology and Philosophy, 20, 365–399.
- Miquel, P.-A., Hwang, S.-Y (2016, this issue). From physical to biological individuation, Progress in Biophysics and Molecular Biology, Available online 16 July 2016, ISSN 0079-6107, doi: 10.1016/j.pbiomolbio.2016.07.002.
- Montévil, M., & Mossio, M. (2015). Biological organisation as closure of constraints. Journal of Theoretical Biology, 372, 179-191.
- Montévil, M., Mossio, M., Pocheville, A. & Longo, G. (2016, this issue), Theoretical principles for biology: Variation, Progress in Biophysics and Molecular Biology, Available online 13 August 2016, ISSN 0079-6107, doi: 10.1016/j.pbiomolbio.2016.08.005.
- Moreno, M., Mossio, M. (2015). Biological autonomy. A philosophical and theoretical enquiry. Dordrecht: Springer.
- Moreno, A., & Ruiz Mirazo, K. (1999). Metabolism and the problem of its universalization. BioSystems, 49, 1, 45-61.
- Morten, E. et al. (2012). The half-life of DNA in bone: measuring decay kinetics in 158 dated fossils. Proc. R. Soc. B. 279, 1748, 4724 - 4733.
- Moss, L. (2003). What genes can’t do. Cambridge, MA: MIT Press.
- Mossio, M., Bich, L. (2014). La circularité biologique: concepts et modèles. In: Varenne, F., Silberstein, M., Huneman, P., Dutreuil, S. (Eds.), Modéliser & simuler. Epistémologies et pratiques de la modélisation et de la simulation, vol. 2. Editions Matériologiques, Paris, 137-170.
- Mossio, M., Saborido, C., & Moreno, A. (2009). An organizational account of biological functions. British Journal for the Philosophy of Science, 60, 813–841.
- Müller, J. (1837-40). Handbuch der Physiologie des Menschen für Vorlesungen. 2 Vols. Coblenz: Verlag von J. Hölscher.
- Needham, J. (1937) Integrative Levels: A Revaluation of the Idea of Progress, Oxford: Clarendon Press.
- Nicolis, G., & Prigogine, I. (1977). Self-organisation in non-equilibrium systems: from dissipative structures to order through fluctuation. New York: Wiley. Noble, 2006;
- Noble, D. The Music of Life. Biology Beyond the Genome; Oxford University Press: Oxford and New York, 2006.
- Nunes, N., Moreno, A., & El Hani, C. (2014). Function in ecology: an organizational approach. Biology and Philosophy, 29, 1, 123-141.
- Perret, N. & Longo, G. (2016, this issue). Reductionist perspectives and the notion of information, Progress in Biophysics and Molecular Biology, Available online 22 July 2016, ISSN 0079-6107, doi: 10.1016/j.pbiomolbio.2016.07.003.
- Peterson, E. (2010). Finding Mind, Form, Organism, and Person in a Reductionist Age, PhD Dissertation, 2 vols., Program in History and Philosophy of Science, University of Notre Dame.
- Piaget, J. (1967). Biologie et connaissance. Paris: Éditions de la Pléiade.
- Pontarotti, G. (2015). Extended inheritance from an organizational point of view, Hist. Phil. Life Sci., 37, 4, 430-448.
- Raj, A. & van Oudenaarden, A. (2008). Nature, nurture, or chance: Stochastic gene expression and its consequences. Cell, 135, 2, 216-226.
- Rao, P.V., Krishna, C.M., Zigler J.S. Jr (1992). Identification and characterization of the enzymatic activity of zeta-crystallin from guinea pig lens. A novel NADPH: quinone oxidoreductase. The Journal of Biological Chemistry, 267, 1, 96-102.
- Rashevsky, N. (1954). Topology and life: In search of general mathematical principles in biology and sociology. Bulletin of Mathematical Biophysics, 13, 317-348.
- Reiss, J.O. (2009). Not by Design: Retiring Darwin’s Watchmaker. Berkeley and Los Angeles: University of California Press.
- Rosen, R. (1991). Life itself. A comprehensive enquiry into the nature, origin and fabrication of life. New York: Columbia University Press.
- Ruiz-Mirazo, K., Etxeberria, A., Moreno A., Ibáñez J. (2000). Organisms and their place in biology. Theory in Bioscience, 119, 209-233
- Ruiz-Mirazo, K., Moreno, A. (2012). Autonomy in evolution: from minimal to complex life. Synthese, 185(1), 21–52.
- Ruiz-Mirazo, K., Moreno, A. (2004). Basic autonomy as a fundamental step in the synthesis of life. Artificial Life, 10, 3, 235-259.
- Saborido, C., Mossio, M., & Moreno, A. (2011). Biological organization and cross-generation functions. The British Journal for the Philosophy of Science, 62, 583-606.
- Shyer, A.E., Tallinen, T., Nerurkar, N.L., Wei, Z., Gil, E.S., Kaplan, D.L., Tabin, C.J., Mahadevan, L. (2013). Villification: How the Gut Gets Its Villi, Science, 342 (6155), 212-218.
- Soto, A., Longo, G., Montévil, M., Sonnenschein, C. (2016, this issue). The biological default state of cell proliferation with variation and motility, a fundamental principle for a theory of organisms, Progress in Biophysics and Molecular Biology, Available online 2 July 2016, ISSN 0079-6107, doi: 10.1016/j.pbiomolbio.2016.06.006.
- Soto, A. Sonnenschein, C. (2005). Emergentism as a default: Cancer as a problem of tissue organization. Journal of Biosciences, 30, 1, 103-18.
- Soto, A.M., Sonnenschein, C., Miquel, P.A. (2008). On physicalism and downward causation in developmental and cancer biology. Acta Biotheoretica, 56, 257–74.
- Thompson, D. W. (1917). On Growth and Form. Cambridge University Press.
- Thompson, E. (2007). Mind in life. Biology, phenomenology, and the sciences of mind. Cambridge, MA: Harvard University Press.
- Van Fraassen, B. (1989). Laws and Symmetry. Oxford University Press.
- Varela, F. J., Maturana, H., & Uribe, R. (1974). Autopoiesis: the organisation of living systems, its characterization and a model. BioSystems, 5, 187–196.
- Varela, F. J. (1979). Principles of biological autonomy. New York: North Holland.
- Wang, B., Hurov, K., Hofmann, K., Elledge, S.J. (2009). NBA1, a new player in the Brca1 A complex, is required for DNA damage resistance and checkpoint control. Genes Dev., 23, 729–739.
- Weiss, P. (1963). The cell as unit. ICSU REVIEW, 5, 185-193.
- Wiener, N. (1948). Cybernetics. MIT Press, Cambridge, MA.
- Wolfe, C. (2010). Do organisms have an ontological status? History and Philosophy of the Life Sciences, 32, 2-3, 195-232.
- Wolfe, C.T., Terada, M. (2008). The ’animal economy’ as object and program in Montpellier vitalism. Science in Context, 21, 4, 537-579.
- Woodger, J.H. (1929). Biological Principles. A Critical Study. London: Routledge and Kegan.
Footnotes
-
Note that we do not aim to discuss the notion of genetic information here; see Perret & Longo (2016) and Longo et al. (2012a) for a critical analysis.↩
-
The notion of “theoretical determination” should not be confused with “determinism”. Determinism corresponds to the assumption that the perfect knowledge of a given situation at a given time entails its future descriptions. Theoretical determination is the framework for understanding the changes of the intended object, and this framework can be not deterministic, as is the case in quantum mechanics, for example. Genocentrism rather corresponds to an assumption of “completeness” of the DNA as a code for development.↩
-
This perspective broadened theoretical determination proper to genocentrism, although it mostly continued to attribute a central role to genes in ontogenesis. In short, “stochastic gene expression” is an increasingly relevant perspective, which modifies the role of randomness in molecular biology, as this moves from “noise” to a form of “functional randomness”, while preserving the genocentric perspective. In Montévil et al. (this issue), we further discuss this issue and show how our analysis of organismal constraints may also propose a tentative understanding of the role of genome and the way its stochastic expression is canalized within and by the organism.↩
-
Our understanding of the concept of gene converges with what Lenny Moss (2003) labels “Gene-D”, the developmental resource that templates amino acid sequences for proteins. “Gene-D” is in contrast to the “Gene-P”, the preformationist gene concept, which serves as an instrumental predictor of phenotypic outcomes.↩
-
Gilbert and Sarkar refer to vitalism as the typical example of a non-naturalist perspective. In fact, although this understanding of vitalism has become quite typical in the 20th century (see also section 2.1 below, about Bertalanffy’s vision), it does not apply to all vitalist accounts. As a matter of fact, some of the most important vitalist schools, such as that of Montpellier in the 18th century (Wolfe & Terada, 2008), explicitly reject and criticize the appeal to non-natural entities to explain living phenomena (see Cimino & Duchesneau, 1997).↩
-
The term ‘organicism’ has begun to be used in its contemporary meaning around the end of 19th century (Peterson, 2010). Its use in relation to previous authors is therefore somewhat questionable. ↩
-
The generative nature of closure seems to adequately encompass one of the main differences between biological systems on the one hand, and artifacts and other categories of natural systems on the other. Intuitively, it seems correct that those situations in which the existence of the parts depends on that of the whole system are indeed characteristic of biological organisms. The parts of a rock do not dissolve if the whole is broken into pieces, just as the components of a computer do not disintegrate if the whole machine is disassembled.↩
-
Indeed, Bernard is rather mentioned for his emphasis on the constancy of the internal milieu than for the vindication of the organizational nature of biological systems. Actually, as is often recalled, his work paved the way for the development of the idea of homeostasis by Cannon (1929) and, later on, by First-Order Cybernetics (Wiener, 1948; Ashby, 1956). Homeostasis, however, designates a general systemic capacity that does not specifically apply to biological systems. In this paper, hence, we do not discuss it. See Mossio & Bich (2014) for additional remarks on this point.↩
-
Without a precise characterization, the idea of a thermodynamically open system in which the parts depend on each other for their own maintenance does not seem to apply distinctively to the biological domain. Let us mention an example that is frequently referred to in this kind of debate, namely, the hydrologic cycle. In this case, a set of water structures (e.g. clouds, rain, springs, rivers, seas, etc.) generate a cycle of causal relations in which each contributes to the maintenance of the others, and is in turn maintained by the others. Clouds generate rain, which (contributes to) generates a spring, which gives rise to a river, which (contributes to) generates a lake, which regenerates clouds, and so on.↩
-
Let us consider an abstract mapping f between the sets A and B, so that f: A = ≫ B. If we interpret the mapping in causal terms, and look for the causes of B, Rosen claims (and develops a detailed conceptual and formal justification that we will not repeat here) that A is the material cause of B, while f is the efficient cause.↩
-
Kauffman has proposed retrieving the classic idea of “work cycle” (in the sense of the Carnot machine), and to apply it in the context of self-maintaining biochemical reactions. Kauffman’s approach is based on Atkins’ ideas about work, conceived as a “constrained release of energy” (Atkins, 1984).↩
-
We note CA → B those aspects of C which play a role in the above asymmetry between A → B and AC → BC at time scale τ. In what follows, we generically use the notation C instead of CA → B whenever this does not give rise to confusion.↩
-
As we will discuss below, the relationship exists also the other way around, the vascular system being dependent on the gut. That is precisely what the principle of organization is about. ↩
-
In organs, repair means replacement of cells by new cells. Accordingly, we consider that “reconstruction” (with variation) is more appropriate than “repair” into a previous state. ↩
-
The relationship of dependence that is relevant for biological closure is a direct one. i.e. a situation in which, considering the different processes that occur at τ2 and contribute to maintaining a relevant aspect of C1 that depends on C2, none of them follows the one constrained by C2, in physical time. This specification is necessary because the definition given above would otherwise apply to a wide range of relationships between constraints, including those in which the enabling and dependent constraints are linked through very long chain of processes. In this case, the concept of dependence would include many biologically irrelevant situations.↩
-
See Montévil & Mossio (2015) for additional specifications of this definition (which are not required for the purposes of this paper).↩
-
This distinction between “constitutive” and “non-constitutive” constraints relies mainly on the definition of dependence established in the previous section. In fact, most external constraints do have causal interactions with the organism and, consequently, either affect it or are affected by it. Yet, even when it can be shown that a non-constitutive constraint interacts with the organism (in which case one may wonder whether or not it is subject to its closure), it should also be shown that, in accordance with the definition, the relationship of dependence is generative (and not only modulatory) direct and, moreover, concerns the relevant aspects as a result of which the entity satisfies the definition of constraint, at the relevant scale.↩
-
A logical distinction could be made between stability, understood as “maintenance through time”, and regularity, which would rather refer to the “synchronic similarity”, for instance, between individuals of the same species. We will not elaborate here on these distinctions and will just focus on stability. It is worth mentioning that both notions can be understood in the light to the idea of genericity of the object, as opposed to its specificity. See Montévil et al. (2016) for details.↩
-
More generally, the principle of organization induces a departure from what could be dubbed a “biophysical posture” in biology, i.e. the idea that biological systems could be understood through the elaboration and composition of local models of functions and dynamics. Rashevsky (1954) has put strong emphasis on the importance of such a departure when he advocates the establishment of what he labels relational biology: “ We must look for a principle which connects the different physical phenomena involved and express the biological unity of the organism and of the organic world as a whole”. In accordance with an organicist perspective, the principle of organization does put the emphasis on the (mutual) relations between the parts, and not on their features considered in isolation. ↩
-
It is important to distinguish between the stability of biological phenomena and their generation. In this paper, we deal with the role of organization in understanding how biological phenomena are maintained through time, and not how they originated. The two issues are of course related and, as a matter of fact, there is a rich literature advocating an organization-centered view on the origins of life. Yet, we hold that the two issues can be treated separately.↩
-
“I mean by this expression that the whole organization is so tied together during its growth and development, that when slight variations in any one part occur, and are accumulated through natural selection, other parts become modified” (Darwin, 1909-1914).↩
-
These preliminary considerations would require a much more full-fledged analysis, which we leave for a future work. In Montévil et al. (2016), we mention the connection between the default state and the other theoretical principle that we propose for biology, i.e. variation.↩